
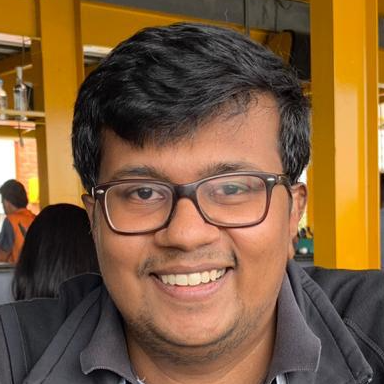
Vasudevan Mukunth is the science editor at The Wire.
Both the LHC and the LUX dark-matter experiment have had their hopes – of finding a new particle – dashed despite being state of the art. This is still good because the rigour of their searches provides hope.

From not being I shall not allow you to say or to think: for not to be said and not to be thought
is it that it is not. And indeed what need could have aroused it
later rather than before, beginning from nothing, to grow?
– On Nature, Parmenides, fragment 6.
Caution: Deceptively complex charts ahead
When an important experiment conducted to find something checks back with zero results after having run for a long time, what can we learn from it?
This is the story of two experiments in high-energy physics.
The first is the search for dark matter, the mysterious substance said to comprise 27% of all matter in the universe. The substance’s name comes from the fact that it doesn’t interact with electromagnetic radiation, rendering it dark and out of sight of (most of) the means by which humankind observes the universe. On July 22, the Large Underground Xenon (LUX) experimental collaboration, South Dakota, reported that its scan of a particular range of energies for dark matter particles had come up blank. LUX is the most sensitive experiment of its kind in operation, and if it came up blank, then theoretical physicists have something to think about.
A relevant ‘actor’ here is the parameter space. The LUX experiment looks for dark matter particles – technically called weakly interacting massive particles (WIMPs) – by paying very, very close attention to a tank filled with liquid xenon. If a WIMP interacts with a xenon atom, LUX’s sensors will pick up on the effects of the interaction (usually some form of radiation). The various properties of these effects say something about the WIMP that interacted; in turn, the properties are characterised by a set of parameters. All the values that these parameters can take are encapsulated by the parameter space.
In the context of the parameter space, a blank result is actually a positive result. It signifies that the parameters can’t take on the values for which the detector scanned. Such a result can be frustrating, but it is also meaningful because it turns the screws on a theory that works with those parameters, driving it closer to the final values. Assuming there will ever be a non-blank result, but even so.
The second story is of the Large Hadron Collider at CERN, Geneva. On August 5, physicists working with the particle-smasher announced that a particle they thought they had detected in December 2015 had vanished – stopped showing up – when they intensified the sweep for it. It appeared to be weighing about 750 GeV/c2, far heavier than the heaviest known fundamental particle (the top quark, ~173 GeV/c2). Of course, at first sighting, physicists weren’t sure it was a fundamental particle either, but there was hope.
Why? Because particle physicists are eager to find the unknown. They’re up against the limits of their understanding of the universe – and that understanding has some embarrassingly gaping holes in it. The understanding is defined by a clutch of theories called the Standard Model, and it is understood to be complete the way it is because all its predictions have been verified. At the same time, it hasn’t been able to step beyond its predictions to explain other discoveries. For example, why is the Higgs boson so light? (Before its discovery, it was expected to be much heavier.) For another, why is gravity so much weaker than the three other fundamental forces? (By 100 trillion trillion times, to be sure.)
The Standard Model has nothing to say.
So when news of the ‘new’ particle was downgraded to having been a fluke, physicists were dejected. At the same time, the parameter space had been narrowed.
Both LUX and the LHC (rather, its attendant detectors) are experiments looking for something that may or may not exist, and within a space of arguably infinite possibilities – like looking for a wrecked fishing boat in the Atlantic Ocean. You’d have to be incredibly lucky to find it on your first day of looking, only slightly less lucky to have a plank of wood or some other flotsam drift your way, and probably get used to being waylaid by storms. But you’d also have to be incredibly careful about knowing what you’re looking for and ignoring everything else.
The last bit is why physicists have an exact threshold beyond which a result is accepted as fact, and anything behind which isn’t good enough no matter the difficulty in obtaining it: the 5σ (five sigma) significance, in the parlance of statistics.
Consider the LUX experiment, which comprises a giant tank of liquid xenon surrounded by sensors looking for WIMPs to interact with xenon atoms. In the absence of WIMPs, some other particles in the atmosphere will be interacting with the atoms, producing some signals in the sensors. The average variation of the signals in this case is called the standard deviation, and it defines the so-called background. Its symbol is σ.
When a WIMP interacts with a xenon atom, the signal it produces should be more pronounced than this background for it to look valid. How much more pronounced? At least five times more than the background’s standard deviation – so the designation of 5σ. The equivalent odds (of a 5σ signal being a fluke) are 1 in 3.5 million.
This interpretation doesn’t work in isolation, either. There are two kinds of significance: local and global. The local significance denotes the chances of finding a particle at exactly one place in the plot. The global significance denotes the chances of finding such a particle anywhere in the plot.

Finally, there’s the ‘look elsewhere’ effect. As physicist Tommaso Dorigo writes, “You looked in many places for a possible signal, and found a significant effect somewhere; the likelihood of finding something in the entire region you probed is greater than it would be if you had stated beforehand where the signal would be, because of the ‘probability boost’ of looking in many places.” This boost is a result of the ‘look elsewhere’ effect.
Moreover, there are two LHC detectors looking for this particle: ATLAS and CMS. So that’s already four datasets: two each of local and global significance from the two detectors. Because of the way they’re calculated, the significance can’t be combined straight away.
So, the 5σ requirement literally represents the level of rigour by which the particle physics community abides. It is intense; it also smothers any confusion because of the ‘look elsewhere’ effect. To get as strong a signal as it did that led to the discovery of the Higgs boson, the LHC had to perform billions upon billions of collisions. And to do that in turn, the mammoth collider cost over $10 billion to build over 25 years.

This is also the context within which a ‘nothing’ result acquires a positive meaning. To find nothing with great rigour means to have refuted certain theories and ideas with great rigour, to have found impossibility over possibility, and to not have to go back and look for something all over again when a new experiment kicks off. It pays to bear in mind that both LUX and the LHC complex are venturing into the unknown with their experiments.
More than one kind of dark-matter particle has been theorised to exist, all with such different properties than no single detector can look for them all at once. The LHC, similarly, is sweeping a range of energies looking for something likely to be extremely short-lived – even more than the Higgs boson was – and if nothing is found, the machine will have to be upgraded with billions of dollars to make it more powerful. And even then, there’s a long, long way to go.
Particle physics advances only with experimental confirmation of theoretical ideas. And theoretical physics has already been exploring the behaviour of particles at energies of a few million GeVs and beyond. The most powerful accelerator, on the other hand, has just started plumbing tens of thousands of GeVs. A ‘nothing’ result at this stage is frustrating not because we’re at the end of the rope; we’re not. It’s frustrating because nature is not being easy. Then again, it rarely has been.