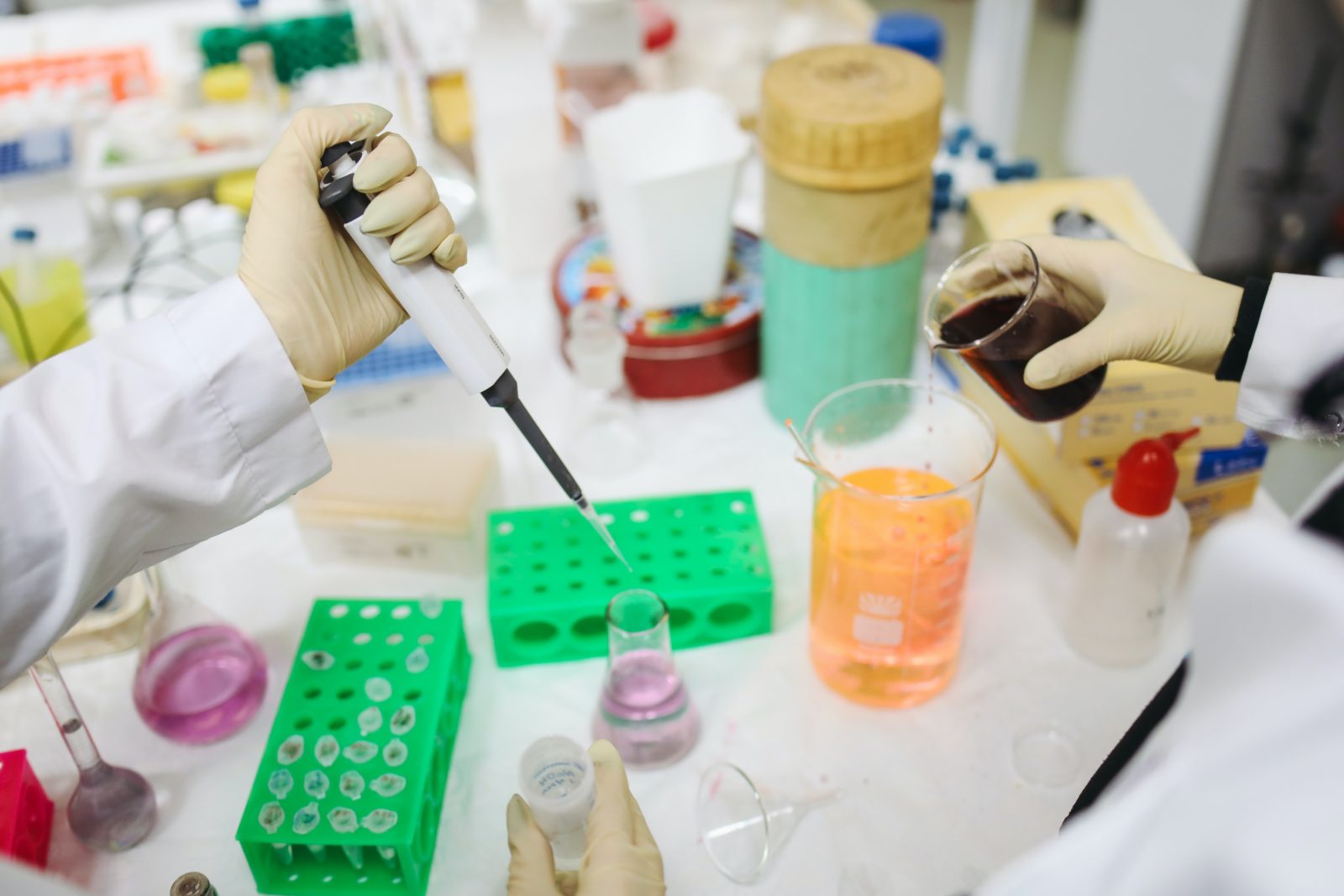
Representative image. Photo: Polina Tankilevitch/Pexels.
The COVID-19 outbreak has pushed hundreds of research labs around the world to engage in problems of disease transmission, drug discovery and vaccine development. As of July 2020, there had been 156 vaccine candidates and 333 therapeutic drugs under development. These are big numbers but all of them are still at different stages of preclinical and clinical trials.
Designing a novel molecule against a target is an exacting process. Therapeutic drugs travel a long journey before they reach the market. All that is disclosed to the public is the status of clinical trials and when consumers can expect to find a drug in drugstores.
Drug design demands complete and clear knowledge about a given disease. Before designing a new drug, scientists study the entire pathophysiology, including aspects of pathogen entry, multiplication in host, symptoms and transmission. The underlying biochemical changes in disease manifestation are focussed in non-infectious diseases. Scientists set up specific checkpoints within a disease cycle and conduct studies to better understand the factors controlling them.
After identifying potential drug targets, scientists then design or repurpose specific molecules from known libraries to competitively inhibit targets and disrupt the disease cycle.
The pathogen that causes COVID-19 belongs to the Coronaviridae family. Members of this family are characterised by their distinctive spike proteins. These proteins allow the virus to attach with specific human cells using ACE2 receptors on the latter’s surface. After attachment, the spikes undergo structural changes to fuse with the human cell’s membrane.
Given the importance of this mechanism, spike proteins have become significant as potential drug targets. Scientists from the University of Texas, Austin, mapped the spike protein’s structure using cryo-electron microscopy. Using their results, other scientists can find suitable molecules to fuse with the proteins and inhibit their ability to attach to human cells.
Designing novel chemicals is time-consuming, so during an emergency, drug-repurposing is a temporary alternative. In this paradigm, researchers investigate already available drugs as therapeutics — like, for example, a research group at IISER Pune has been doing. For another, the 3CLPRO main protease enzyme of the novel coronavirus produces functional proteins from polyproteins, and is responsible for viral replication. Many researchers are trying to find its possible inhibitors among repurposable drugs.
A structure approach to drugs
The case of the novel coronavirus is just an example. The larger point here is about dealing diseases with a structural biology approach. Target-ligand binding and their associated interactions are important aspects of designing drugs. Earlier, scientists used to rely on known chemical reactions to identify and repurpose potential drugs. Today, thanks to advancements in science and technology, they can and do access knowledge of biological structures to accelerate and enhance drug design.
Biomolecules are invisible to the naked eye. In fact, they’re so small that most microscopes won’t do; researchers instead use a different science.
All biological macromolecules are long chains of individual monomeric units. Nucleotides make up nucleic acids and amino acids make up long protein chains. These long chains fold back into three-dimensional structures with specific shapes. These shapes are important to how the proteins function.
For example, enzymes are, biochemically speaking, proteins and perform their function substrates bind with them at an active site. An enzyme won’t work if the wrong substrate binds to it – a feature commonly compared to a lock-and-key system. Similarly, if an enzyme is misfolded or an active site is deformed, it loses its ability to bind to specific substrates and the enzyme can’t function.
In effect, the functional efficacy of enzymes depends on chemical interactions as well as its structure. These aspects matter to structural biologists along with enzyme chemistry itself.
Studying biomolecular structures has become more important to understand disease mechanisms. Structural biology is an interdisciplinary subject that demands knowledge of molecular biology, biochemistry and biophysics. Molecular biology deals with genetic sequences. Biochemistry deals with the biochemical properties of proteins, amino acids, etc. Biophysics uses different techniques like X-ray crystallography, electron microscopy, nuclear magnetic resonance (NMR) spectroscopy and cryogenic electron microscopy to decipher structures using information from biochemistry and molecular biology.
Altogether, structural biology can provide a complete picture of the desired biomolecules.
Deciphering structures
Researchers first clone the genomic stretch of a desired protein into a plasmid and express it to obtain the required quantity of proteins. Then, they isolate the expressed protein, purify it and crystallise it. Next, they use a suitable technique, like X-ray crystallography, to examine its three-dimensional geometry. Which technique is used depends on the molecule to be studied. For example, molecules that can’t be crystallised are studied either with NMR spectroscopy or cryo-electron microscopy.
The first documented attempts to study the structures of biomolecules began in the 1950s, when Max Perutz and his colleagues exposed myoglobin to a beam of X-rays. In 1962, Perutz and John Kendrew were awarded the Nobel Prize in chemistry for their work elucidating the structures of myoglobin and haemoglobin this way. The other techniques, from NMR spectroscopy to cryo-electron microscopy, emerged much later, all of them advancing structural biology in distinct ways.
Perutz’s studies explored the significance of active-site structures, and building on his and others’ work, researchers were able to understand the structure of insulin, chymotrypsin, lysozyme and trypsin by the 1960s. These studies highlighted the role of substrate-binding selectivity and molecular specificity, which are important concepts that link structural biology to drug design. In the 1970s and 1980s, researchers initially focused on clinically important enzymes, before broadening their gaze with time.
Structure-based drug design entered a new era after researchers developed antiviral drugs to treat AIDS. Today, we know the three-dimensional structures of more than 137,000 biomolecules (in January 2018), as archived in the Protein Data Bank. These include different proteins, nucleic acids, their complexes and small ligand molecules. When scientists have developed or elucidated a new macromolecular structure, most scientific journals require the corresponding details to be deposited in the PDB before their paper is published. Researchers and other professionals can freely access the data bank.
Subsequently, advanced biophysical tools and computational techniques like molecular docking and homology modelling boosted the drug-design process. Today, researchers can screen molecules compatible with a specific target site using computer algorithms, then score and rank shortlisted ones based on different parameters, then identify best-fit candidates for biochemical assays, and finally forward them for preclinical and clinical studies.
A structure approach to diseases
The chemistry-oriented traditional drug design process helped us produce powerful broad-spectrum antibiotics capable of fighting a variety of infections. However, these antibiotics could also affect otherwise harmless or beneficial pathogens and cause more harm. Antibiotic resistance is also one of the major crises of the 21st century.
One alternative is narrow-spectrum antibiotics that kill only specific microbes, as well as avoid cross-resistance in non-targeted molecules. We can develop such highly specific drugs by understanding the structures of different biomolecules involved.
Alzheimer’s disease, Parkinsonism, Huntington’s disease and prion diseases are neurological diseases characterised by the presence of amyloid plaque. When misfolded proteins cluster into rigid aggregates resistant to proteolysis, they become plaques. And when plaques are deposited on essential organs, they interfere with important functions and sometimes even cause death.
Parkinson’s patients have spherical masses of misfolded α-synuclein, called Lewy bodies, in neuronal cells in the brain. People with Alzheimer’s disease, amyloid β peptides aggregate into oligomers, protofibrils and fibrils that then tangle around, forming plaques.
A type of protein called tau protein is also known to aggregate in the neuronal cells of those with Alzheimer’s. The tau protein stabilises microtubules in healthy neurons. In Alzheimer’s disease, the tau proteins are not associated with microtubules; instead, they clump with one another and become entangled in the neuronal interior. These tangles interfere with communication by blocking electric signals. The neurons eventually stop functioning and disrupt one’s memory and language and reasoning abilities.
The medication currently available for Alzheimer’s relieves painful physiological symptoms. However, structural studies could help reveal the exact role and mechanism of plaque formation in the disease mechanism, and we can then design suitable drugs to either stop protein clustering or dissolve existing plaque.
Starting from the early X-ray studies in the mid-20th century, the structural approach to studying disease mechanisms and designing drugs has revealed to us a plethora of clinically significant molecules. There’s every reason to believe it can continue to reveal new medical treatments, help improve old ones and improve the quality of our lives.
I.V.S.N. Prathyusha is a science enthusiast and writer.